
This article was part of a project we ran to celebrate the International Year of Astronomy 2009. The project asked you to nominate the questions about the Universe you'd most like to have answered, and this is one of them. We took it to cosmologist John D. Barrow, Professor of Mathematical Sciences at the University of Cambridge, and here is his answer. You can also listen to his answer in our podcast.
Are the constants of nature really constant?
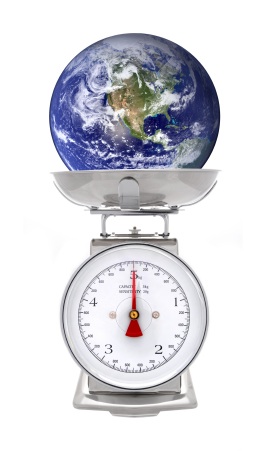
Our units of weight, distance and time depend on human choice and therefore are not universal.
First of all you have to decide what those constants are. If you pick the wrong quantities and believe that they are constants, then the fact that you find them to vary later on won't tell you anything except that you'd made the wrong choice. Physicists have had a long time to try to decide, both observationally and then on the basis of their theories, what things in nature they should regard as absolutely unchanging — the fundamental features of the Universe. These are, perhaps, not some of the things you might have thought.
Who decides what is constant?
We are used to all sorts of constants and standards in every day life, the quality of a rubber tyre, or the standard wire gauge — there are many of these supposedly unchanging quantities in ordinary and industrial life. Most of these grew out of the industrial revolution in the nineteenth century, when there was a huge increase in manufacturing. At that time there was a famous Irish physicist called George Johnstone Stoney, who became the vice-president of the Irish Academy. He was well known for many achievements, in particular for predicting the existence of the electron, and he even gave the name to that particle before it was discovered. Stoney was asked to chair a panel at a British Association for the Advancement of Science meeting to try and decide on a system of units which would handle all the industrial quantities that were springing up at that time. He surprised everyone by coming up with a system which he called natural units.
Planck units
The Planck unit of mass is defined by
Stoney pointed out that we shouldn't worry about things like inches, or metres, or seconds. Rather we should look to the so-called constants of nature, for example the gravitational constant, the speed of light, and of course the constant Stoney himself had introduced, the charge of the electron. Stoney realised that if you juggle these constants around in the right way, you can make quantities that have units of length, mass and time, and these Stoney called natural or fundamental units. These units don't depend on human choice, rather they are defined by the nature of electromagnetism, or of light and gravity. Today we use similar units in physics and cosmology, called Planck units. They're only very slightly different from Stoney's units, but they are based on exactly the same idea.
The constants of nature define what the Universe is like in a way we could communicate to people on the other side of the Universe. If we wanted to tell people in another galaxy how tall we are, and if they, like us were using fundamental units arising out of these constants of nature, then we could all agree on what we are talking about.
Ditch your dimensions
But what one has to worry about today is of course whether those quantities like the speed of light, the charge of an electron, Planck's constant, or the mass of the electron, really are constant. All these quantities have units. The unit of the speed of light is distance per time, i.e. metres per second. The mass of the electron is measured in grams, and so forth. If you ask whether those quantities change in time you have to worry about the units in which you are measuring them. If all speeds are changing, or all lengths are changing, or all times are changing, then it might be rather hard for you to decide whether the quantities measured relative to these speeds, lengths and times are also changing. So in practice it's a good idea to focus attention on what physicists call dimensionless constants — quantities that don't have any units.
For example, instead of the mass of the electron, you could look at the ratio of the mass of the electron to the mass of the proton. That is just a number, because the units cancel out — it's around 1/1836. Similarly in electricity and magnetism there is the dimensionless fine structure constant, which describes the strength of electromagnetic interaction. Gravity also has a dimensionless constant, called the gravitational coupling constant, which describes gravitational attraction. These unusual dimensionless numbers are what defines our Universe. They make it different from other Universes we could imagine.
Why might the constants not be constant?
One of the great paradoxes of theoretical physics is that although we can measure the constants of nature to a great degree of accuracy in experiments, we have no idea why they take the values they do. Some people hope that one day we will find a great theory of everything, which will uniquely and completely specify all these numbers by showing that for our Universe to exist they must take the values they do. No other Universe is possible. I think that up to the early 1980s most people expected that such a theory would be found, certainly Einstein expected it, and he worked quite hard to develop such a theory himself in the last part of his life.
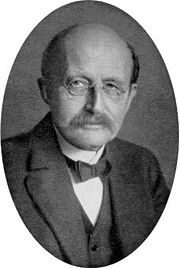
The German physicist Max Planck gave his name to the most fundamental units of mass, length and time in physics.
However, developments in string theory have shown that many of the constants of nature could take a range of different possible values, without causing any contradictions in the theory. There could be universes where the constants take different values to the ones we see in our Universe. These universes would satisfy the same laws of nature, only the forces would have different strengths and some might not even exist. For example, in string theory you have theoretical universes with only a force of gravity, or only gravity and electromagnetism, but no radioactivity and no nuclear forces. Or you might have all the forces we see but with rather different strengths. So far, it seems that there are more than 10500 different possibilities, but we don't yet fully understand if they all really are as possible as they appear. An added subtlety is that in most of these possible worlds life cannot exist.
The other odd thing about string theory is that it seems to require the Universe to have many more dimensions than the three we walk around in. The only way we can reconcile that prediction with the fact that we do just walk around in three dimensions, is that the other dimensions are fantastically small. But this has an awkward consequence for the constants of physics. If the Universe really does have ten dimensions, seven of them really tiny so that we can't see them, then the real constants of nature are all defined in ten dimensions. The things that we are measuring in our laboratories are then just three-dimensional "shadows" of the real constants. And there is no reason why the shadows should be constant — they're not truly fundamental. If these other dimensions in the Universe were to wobble slightly, or just a bit bigger, we would notice that because our three-dimensional constants would change at the same rate.
All this means that some years ago there was motivation for studying whether the constants might be varying. Back in 1999, the astronomer John Webb, of the University of New South Wales, Sydney, and I decided to initiate an observational programme to try to test whether some of these constants were indeed varying very slowly. There were then new detectors on the best telescopes in the world which had a light detection sensitivity at certain wavelengths that was better than that of any laboratory experiment. So astronomy provided a better way to test whether the constants were really constant than any laboratory experiment — this is rather surprising!
Measuring if constants are constant
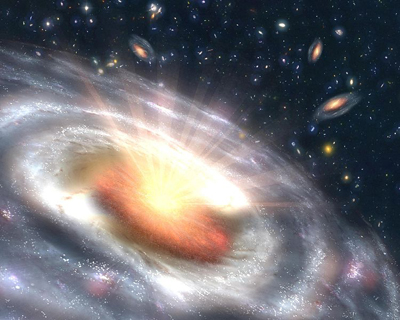
An artist's impression of a quasar. Image courtesy NASA.
Over many years we and our collaborators looked at the spectra of light from distant quasars. We picked quasars because they are the brightest objects we can see in the distant Universe. They are very far away, which means that the light we now see from quasars has spent many billions of years reaching us. So when we receive that light and analyse it we are getting a snapshot of what the Universe, including the laws of physics and the constants of physics, was like many billions of years ago.
We developed a new way of analysing the absorption spectra of light coming from quasars when it had passed thought dust clouds. This made it possible to compare features of quasar light to what we saw here in the laboratory. It enabled us to test whether certain constants of nature were the same in the vicinity of a quasar as they are here in the laboratory. We did this over many years for hundreds of objects and so obtained a very large data set. To avoid a bias being created by a certain technique or instrument, the data was taken by different observers on different telescopes and then mingled together.
A non-constant constant?
We were particularly interested in the fine structure constant, which measures the strength of electromagnetic interaction. Our experiment did indeed pick up a tiny increase of about six parts in a million over 13 billion years in the value of the fine structure constant, a change that is too small to be detected in a lab experiment. As a result, Håvard Sandvik, Joao Magueijo and I thought about what a Universe with a slowly changing fine structure constant would look like, and found that there would be consequences for gravity.
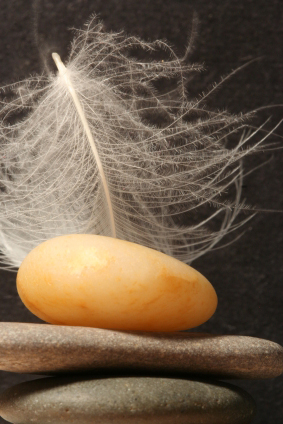
In a vacuum a feather accelerates at the same rate as stones do.
One of the laws of physics, known as the weak equivalence principle says that objects accelerate at the same rate in a vacuum, irrespective of their composition. But it turns out that if the fine structure constant is changing very slowly, then the weak equivalence principle isn't quite true: there will be a tiny difference in the way an object made from iron will fall in a vacuum compared to one made out of carbon or some other material. If the fine structure really is varying as detected in our experiment, then this difference is predicted to be one part in 1013. That is incredibly small. We can't detect such a small difference on Earth because we run into fundamental limits of experimentation (though we are not far off). To get the extra accuracy we need to go back into space. There are currently several proposals for space experiments that would seek to detect a violation of the weak equivalence principle, including one called STEP with an expected accuracy of about one part in 1016, but they are still waiting to be funded.
In any case, here we have an interesting example of how a variation in a constant that is just about electricity and magnetism ends up having consequences for gravity which you might not have suspected.
So are the other constants changing too?
At some level you can never know for sure. There could always be variations that are smaller than what can be detected by the most accurate experiment you can do. But the variations that we seem to have been seeing in quasars will probably be confirmed or refuted soon. Using new atomic clock experiments and new astronomical detectors, we will find out whether these results are real, or whether there is something else going on that mimics the effect of a variation in the fine structure constant near quasars.
John Webb and his team have also been analysing a big new set of quasar spectral data that has been gathered over the last two years — maybe by the end of this year there'll be more results. Other observers are interested in the electron/proton mass ratio, about which it is easier to obtain evidence (also from quasar spectra), and they are closing in on determining if this is varying to the same level of accuracy.
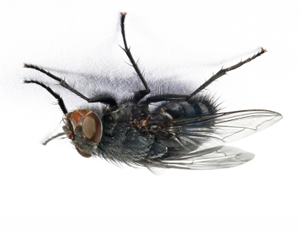
Gravity is so weak that flies can walk on the ceiling.
Something like a variation of the gravitational constant, however, is much more difficult to determine. If you look at the back of your physics text book for the values of the constants of nature, you'll see that lots of them, for example the charge of the electron, or the fine structure constant, are determined to a huge number of decimal places. The exception which sticks out like a sore thumb is the gravitational constant. It is very poorly determined because gravity is so weak on the scale of the laboratory. The fact that a fly can walk around on the ceiling, and that you can suspend a heavy mass with a magnet shows just how weak gravity is. The inaccuracy in our determination of the value of the gravitational constant means that we are also very poor at determining whether it is changing.
Moreover, as I said at the beginning, we don't have any theories about why the constants of nature have the values that they do. So we're missing a very important ingredient in the story. I suspect it is only when we know why they have the values that they do, or whether any of the values are actually randomly determined by events during the early stages of the Universe, that we will be able to say if we should expect any of them to change.