
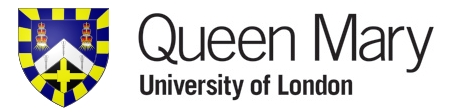
This article is part of the Researching the unknown project, a collaboration with researchers from Queen Mary University of London, (QMUL) bringing you the latest research on the forefront of physics. Click here to read more articles from the project.
In tackling two of the most important questions in physics today the research conducted at QMUL is helping to piece together the creation story of the Universe. The research looks into the bizarre world of particles called neutrinos.
What are neutrinos?
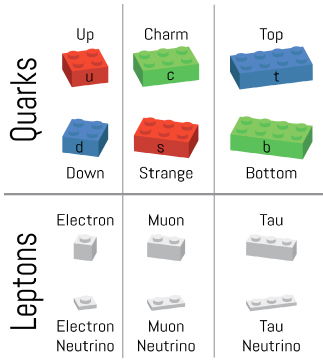
The elementary particles are the building blocks of nature. Image: Ben Still.
When you split nature apart into its smallest parts, like breaking up a LEGO model, you get to a point where it cannot be broken up any further. With a LEGO model you end up with the individual bricks and with nature you end up with the elementary particles. Everything we can see in the Universe around us is made up from some collection of these particles.
In the same way as LEGO bricks can have different shapes, sizes and colours the particles also have different properties associated with them. They do not have size, shape or colour to speak of, but instead their properties are defined by things such as their mass, how they interact with the forces of nature, and how they can combine to form larger composite particles.
Most particles have an electric charge. All particles with an electric charge interact by being attracted or repelled by other electrically charged particles. It is the electrically charged particles that combine to form atoms of the chemical elements.
Some elementary particles, however, do not have an electric charge. These particles do not make atoms and rarely interact with the other particles. Their lack of interaction makes them very ghostly and rare to detect. These elementary particles are called neutrinos. Despite their feeble behaviour it is thought that they might hold answers to some of the biggest questions in physics today.
The problem with antimatter
We suppose that the Universe began with an explosion of pure energy known as the Big Bang. From the pure energy, matter particles were created alongside so-called antimatter particles. Matter makes up everything we see around us, from the Earth to the most distant star. Antimatter, however, seems to only be created where there is lots of energy, for example in particle physics experiments or at the edges of black holes. Particles and their anti-particles are total opposites of each other in every way. For example, a negatively electrically charged electron has a positively electrically charge antimatter version which we call the positron.
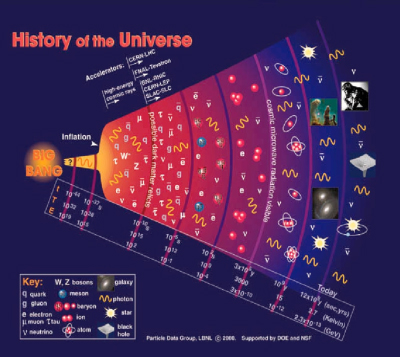
This beautiful poster depicting the history of the Universe was produced by Particle Adventure.
From Einstein's famous equation E = mc2, where E stands for energy, m for mass and c for the speed of light, we know that energy and mass are related. In fact, particle physics experiments can transform one into the other. Each time mass is produced from energy, equal amounts of matter and antimatter are seen to be produced. When matter and antimatter meet each other they annihilate to form pure energy. And since matter and antimatter are opposites, they attract each other and so would inevitably meet.
All of this is not good for the idea of a Big Bang birth of the Universe. From a pure energy Big Bang there would be lots of matter and antimatter created when the Universe was young. Given time, certainly by now, all of the matter and antimatter would have annihilated to form pure energy once more. If the balance between matter and antimatter were perfect then our Universe today would be just energy in the form of microwaves and a lot of neutrinos — and you can't build an atom from those.
How much does nature prefer matter to antimatter?
When we write down the forces of nature and the properties of the particles in the language of maths, we use the concept of symmetry. A symmetry is anything that takes an object, manipulates it and returns that object to its original state. A example of an object is a square. A manipulation that leaves a square identical is a rotation through 90 degrees — you'll find yourself back with the same picture of the square. You could do this all day long and get the same result. This is an example of a rotational symmetry. You could also reflect the square in a mirror and the mirror version of the square would be identical to the original. A combination of rotation through 90 degrees and a reflection would also return to the same square. Any manipulation or combination of manipulations like this is called a symmetry.
The symmetry that exists between matter and antimatter, which turns energy into equal amounts of each in our experiments, is called charge-parity or CP symmetry. Instead of rotation or mirroring, it is the manipulation of mathematical equations that change matter into antimatter in the theory.
Let's draw a picture to represent the Universe with equal amounts of matter and antimatter, as is seen in experiments. The picture below will do, it is based on a tessellation from my favourite artist M.C. Escher. In this picture of the Universe we use the white flying fish to represent matter and the black flying fish to represent antimatter.
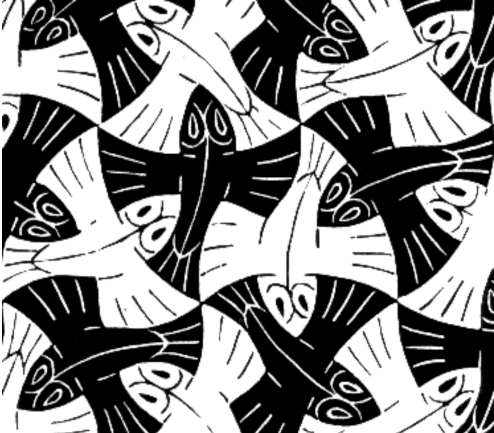
Matter and antimatter are opposite in every way including having opposite electric charges (if they have them!). Electric charge in this picture is represented by the colour (white or black). Our first manipulation is turning all black to white and white to black. This manipulation changes the electric charge of every point of our flying fish Universe. This manipulation is itself not a symmetry as now every point in the picture has become the opposite of its original colour (charge).
To get back to our original picture we need to combine this with another manipulation, parity. Parity changes the direction of space, similar to the way that left seems to become right when we look into a bathroom mirror. If we not only mirror our picture so that left becomes right and right becomes left, but also so that up becomes down and down becomes up, we get back to our original picture of the Universe. These combined transformations of charge and parity, which have brought us back to our original picture, are the CP symmetry mentioned above. They are exactly the symmetry that is used in the mathematics to describe the balance between matter and antimatter.
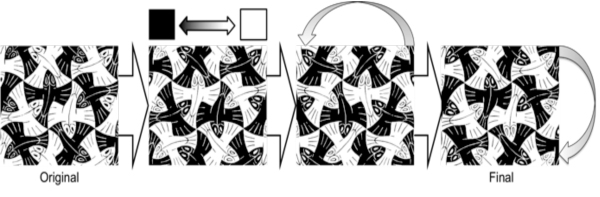
Those with the best eyesight out there might have noticed that we are not quite back to the same Universe. Although on the whole the picture looks the same, there are differences when looking at the finer detail. The eyes of the fish are a different colour. This is exactly how we think our Universe works. On the whole, day to day in experiments, we see the larger picture of a perfect balance between matter and antimatter. But with time we can start to see the finer detail and we start to see an imbalance. The imbalance in this picture of the Universe is far greater than the imbalance we find in our Universe, which is around 1 in 10 million. If we did represent that in our picture it would be just one pixel; even those with the best eyesight would probably never spot that!
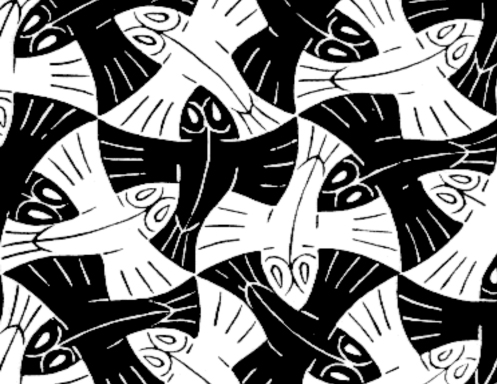
Measuring the asymmetry between matter and antimatter has been the goal of many experiments. The ATLAS, CMS and LHCb projects at the Large Hadron Collider at CERN in Switzerland and their predecessors have seen an imbalance using all particles with an electric charge. The imbalance these experiment see is however far too small to account for all of the matter that make all of the atoms today. This is why we focus on the finer detail of the neutrino and its behaviour.
How neutrinos can help
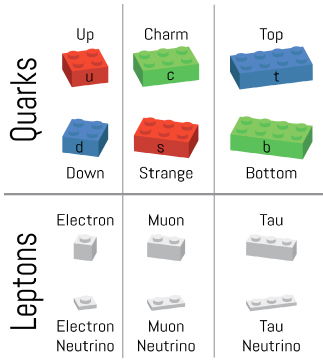
The elementary particles are the building blocks of nature. Image: Ben Still.
The imbalance between matter and antimatter has been seen in particles called quarks (see the image on the right) and it possibly exists between the charged leptons (the electron, muon, and tau) and neutrinos. It is hoped that in looking at the leptons the desired amount of bias for matter is seen to agree with current scientific theories of the creation of the Universe. But we are yet to accurately understand the everyday behaviour of neutrinos. Neutrinos come in three different types: they can be electron-like, tau-like or muon-like. One curious feature is that they can flip between the three types as they travel over large distances. The reason for doing this is linked to their ghostly nature and the fact that they have a tiny, but non-zero, mass. It was first determined that neutrinos behave this way in 1998 and was the first physics beyond the otherwise incredibly successful standard model explanation of particle physics.
Although the theory behind this flip is well understood the extent to which they choose to flip is something that has to be measured through experiment. The T2K (Tokai to Kamioka) experiment, on which QMUL researchers are working, is using a man-made neutrino beam to look at the probability that neutrinos flip from one type to another. T2K scientists have for the first time seen muon-like neutrinos flipping into electron-like neutrinos. This observation is one of the last pieces of the puzzle required before tackling the difference between matter and antimatter with neutrinos and answering the biggest question in the scientific creation story of our Universe.
So next time you are talking particle physics with friends (and who wouldn't) don't forget the humble neutrino. The experiments may not be the biggest or involve the highest energies, but they are answering some of the biggest questions out there — and QMUL is at the forefront. The next ten years are promising to be exciting with each year providing lots of new knowledge about neutrinos and the secrets they may hold. Who knows, they might even prove that the most popular theories are incorrect!
About the author
Ben Still is a research associate at Queen Mary University of London working in the field of particle physics. He spends his time on an experiment called T2K which fires neutrino particles 295km across Japan from East to West. Why? All in an attempt to understand how the behaviour of neutrinos contributed to the creation of the Universe in the first few seconds after the Big Bang. Aside from research Ben has worked with installation designers, artists, school teachers, students and others in developing many ways to communicate particle physics and science as a whole. For this communication work Ben was recently awarded the Institute of Physics High Energy Particle Physics Science in Society prize.
Comments
neutrino mass
My problem with the neutrino mass issue is that we believe neutrinos (in their ghost phase) travel at the speed of light (re: Super Nova 1987 A) but when these neutrinos morph into masslike objects, do they retain the superluminal speed? Or do the bump into the light-speed problem, in which case, we should see some Cherenkov radiation.
Comment please