
This week CERN announced that the Large Hadron Collider (LHC) will run until the end of 2012, rather than being shut down earlier for an upgrade, as had been planned. "If LHC continues to improve in 2011 as it did in 2010, we've got a very exciting year ahead of us," said CERN's Director for Accelerators and Technology, Steve Myers. "The signs are that we should be able to increase the data collection rate by at least a factor of three over the course of this year."
So when can we realistically expect the first ground-breaking results to come through? Last week, John Ellis, former leader of the theory division at CERN, addressed an audience of physicists at the University of Cambridge to update them on the current state of play. Plus went along and also managed to catch Ellis for a quick interview.
The Higgs boson
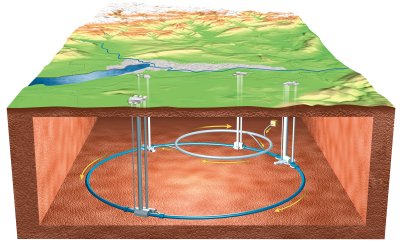
A diagrammatic view of the LHC. Image © CERN.
One mystery the LHC will almost certainly clear up is why things have mass. The standard model of particle physics, which describes the fundamental particles and their interactions, has been remarkably accurate in its predictions, but it contains a major flaw: it says that fundamental particles should have no mass, but physicists have known for a long time that some of them do.
A possible resolution of this puzzle is based on an approach developed in the 1960s by the physicist Peter Higgs. Higgs proposed that space is filled by a mysterious force field, now known as the Higgs field. Unlike the more familiar fields associated to gravity and electromagnetism, the Higgs field is exactly the same throughout space, it doesn't have a particular direction associated to it and it's been around since the beginning of the Big Bang.
Ellis likens the Higgs field to a snowfield which extends infinitely and uniformly in all directions. "Imagine you're trying to cross this field. If you've got skis on, you just skim across the surface and travel very fast. If you're wearing snowshoes, you sink in and travel more slowly. And if you've got no equipment at all, you sink in deeply and travel very slowly." Similarly, different particles interact differently with the Higgs field and their interaction determines how fast they travel. Those that are held back by the field are experiencing inertia, and according to Newton, this means that they have mass. Exactly how strongly particles interact with the Higgs field is unclear, but at least the idea solves one piece of the mass puzzle.
Now quantum physics tells us that we can also think of force fields in terms of particles. Ben Allanach, a theoretical physicist at the University of Cambridge, describes the Higgs field as a weird sort of quantum jelly that extends through space. "Like any liquid, the jelly can be calm like the surface of an undisturbed lake, or it can ripple. A ripple would look like a particle in experiments — this particle is the notorious Higgs boson."
Physicists hope that the high energies created at the LHC will produce the Higgs boson. "In particle colliders, Einstein's most famous equation, E=mc2 is in action," explains Allanach. "The idea is to turn the energy E of the particle beams into a mass m of the exotic particle (for example the Higgs boson) that one wants to produce."
Encouragingly, experiment and theory both suggest similar masses for the Higgs boson, somewhere in the region of 120 GeV (gigaelectronvolts). "The next question is how easy it would be to find a 120 GeV Higgs boson at the LHC," says Ellis. "The answer is that this isn't the easiest [of situations], a heavier particle would be easier to detect because it has a higher probability of decaying into a final state that is easy to see. But it's not impossible to see a 120 GeV Higgs." Ellis thinks that by the end of 2012 we will know, via LHC experiments, whether a Higgs boson with a mass in the required region exists.
And if it doesn't turn up? "We'll have to go back and come up with a more complicated theory," says Ellis. "Maybe the Higgs boson is not as we expected or there are more than one Higgs boson. But I'm optimistic that within the next couple of years, if we're not absolutely sure that there is a Higgs boson, we'll at least have some pretty solid indications."
Dark matter
The LHC may also shed light on the dark matter which physicsts believe makes up 96% of the matter that's out there. Unlike the ordinary matter we can see, dark matter doesn't emit any electromagnetic radiation — light — and so we can't detect it by conventional methods. We know that it exists because of its effects: the celestial bodies we observe respond to gravitational forces much greater than those accounted for by known matter.
Find out more in our article What is dark matter?
But physicists do have some idea of what dark matter could be made of. In the 1970s theorists developed the notion of an exotic kind of symmetry, called supersymmetry, which they believe is present in the structure of spacetime. It has never been observed, but if it does exist, then ordinary particles have invisible cousins, their so-called superpartners. According to most supersymmetric theories (there are several), the lighter supersymmetic particles are stable: they don't decay into anything else. This means they have been around since the Big Bang which makes them candidates for dark matter.
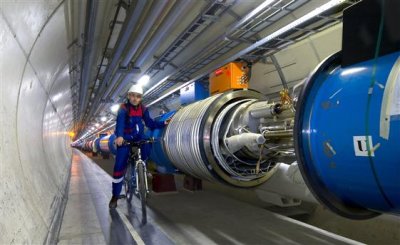
Exploring the Universe at the LHC. Image © CERN.
If superparticles do indeed exist, then they should be produced in the proton collisions at the LHC. They'll of course be invisible, but we may be able to detect them by looking closely at what happens after a collision. Ellis uses a billiard ball analogy. After colliding, billiard balls will move off in opposing directions because momentum needs to be balanced: if one ball moves off to the left, then the other moves off to the right. "Now if you have invisible particles which carry off momentum without being seen, then that would look a lot like colliding two billard balls and both going off to the right because there's an invisible third billiard ball, or even a fourth one," explains Ellis. "So the way to look for invisible particles is to look for collision events with missing momentum in the transverse direction to the one the protons are travelling in."
Two LHC experiments (CMS and ATLAS) have already released the results of initial analyses of data that may reveal such asymmetric collisions. "One can expect more complete analyses of 2010 data soon, and the 2011 data should provide much greater sensitivity," says Ellis.
String theory
You can read more about string theory in our articles From Newton to Einstein and beyond and Tying it all up.
Supersymmetry is related to another entirely theoretical construct called string theory, which was designed to solve the biggest unsolved problem in modern physics: the fact that the theories which describe the world at atomic and sub-atomic scales cannot account for the force of gravity as described by Einstein's theory of general relativity. What's needed is a unified theory, but this has so far proved ellusive. String theory is the strongest contender, but to date string theory remains purely theoretical — it cannot be tested directly in the lab and its exact relation to other areas of physics is unclear.
But there are indirect ways of testing string theory. One comes from the intriguingly named holographic principle, which links string theory to the more conventional quantum field theory that underlies the standard model of particle physics. The principle provides a precise mathematical correspondence between the two theories, which allows you to translate some predictions of string theory into measurable phenomena.
To find out more about the holographic principle, read our article The illusory Universe.
An example is the quark-gluon plasma, an extremely hot and dense soup of particles which physicists believe filled the early Universe for just a few microseconds after the Big Bang. String theory, via the holographic principle, provides predictions about the properties of this mysterious substance. In particular, it predicts that the viscosity of the plasma could be a lot lower than that of any other known fluid.
Using heavy ion collisions, physicists have managed to recreate the quark-gluon plasma in particle accelerators and they have indeed measured a very low viscosity. If these measurements can be confirmed and improved at the LHC, then this may provide the first glimpse of evidence that string theory may indeed be correct. "The holographic principle has a firm theoretical basis and there are real prospects for experimental advances in the coming years," says Ellis.
Another approach for testing string theory involves extra dimensions and tiny black holes. According to string theory, spacetime contains an extra six dimensions beyond the three dimensions of space and one dimension of time we can perceive. These extra dimensions, so string theorists believe, are curled up so tightly that we just can't see them. They might also explain why gravity is quite weak compared to the other forces: some of its effect may be active in the extra dimensions and therefore lost to the ordinary four-dimensional world.
Read more about string theory and its extra dimensions in Hidden dimensions.
The high-energy environment produced in the LHC may enable the gravity that's usually trapped in the extra dimensions to affect the colliding protons. Gravity would then become sufficiently strong for microscopic black holes to appear for fleeting moments. But these would be different from the large black holes we observe in astrophysics. In particular, they would not emit the so-called Hawking radiation in quite the same way as big black holes do. And this difference is something that can be measured at the LHC. "If you saw any deviation [from the usual emmission spectrum of astrophysical black holes], then that would be a wonderful way of testing modifications to gravity and in particular for testing string theory," says Ellis.
Wonderful it may be, but the approach is also rather speculative. Ellis is unwilling to hazard a guess as to when the LHC is likely to produce firm evidence for string theory. But what about those tiny black holes? If they do appear, what is the chance that they will gobble us all up, as some have suggested? "Zero," says Ellis. "This idea is absolute rubbish." The fact is that the LHC can do nothing that nature hasn't done already, and so far we've survived it all.
Comments
The LHC Can't Do Something that Nature Hasn't Done Already?
I don't know if I necessarily believe that. What about bashing superatoms together?
It's true.
High energy (GeV) cosmic rays do this all the time (with one stationary atom). The difference is that the LHC does it in a controlled environment, so that we can measure said collisions much easier.