What is dark matter?
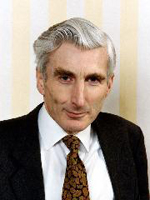
This article was part of a project we ran to celebrate the International Year of Astronomy 2009. The project asked you to nominate the questions about the Universe you'd most like to have answered, and this is one of them. We put it to Martin Rees, Astronomer Royal and Professor of Cosmology and Astrophysics at the University of Cambridge. Here is his answer. The second part of the question has been answered in Plus by John D. Barrow.
What is dark matter?
The galaxies in our Universe are not exclusively made up of the stuff we can see, but are held together by the gravitational pull of so-called dark matter. We shouldn't assume that everything is equally conspicuous and shouldn't therefore be surprised by this discovery.
The image of the Earth at night from space at first glance reveals no discernable pattern, but a closer look discloses many identifiable features — light from major metropolises, oil wells alight in the Middle East, and the warm glow from millions of wood-burning stoves in India. These beacons in turn outline the familiar pattern of the coastlines and continents. But most things on Earth do not shine, and were this the only available snapshot of the Earth, we would have a rather skewed and incomplete picture of the terrain. The majestic mountains of the Andes, Rockies, and Himalayas for instance would be hidden from view.
It is like this when we look outward into the cosmos. Since stars radiate most of their energy as visible light, optical telescopes remain the most powerful probes, however there are several other windows into the Universe, like radio, or X-rays. In fact, prominent objects in the radio sky are quite distinct from those that dominate in optical images.
The evidence for dark matter
Many things that don't shine in visible light can perhaps be detected via other techniques. The electromagnetic spectrum can be divided into about 80 octaves, of which visible light is but one. But what we have discovered is that most things in the Universe neither emit nor absorb any kind of radiation and are completely dark. The evidence for this came first in the 1930s, but was taken seriously only in the 1970s. Galaxies or clusters of galaxies seem to be held together by the gravitational pull of more material than we actually see — that invisible material has been dubbed dark matter and is thought to make up around 25% of our Universe. By observing galaxies in a group and how they are moving around we can then work out how much gravity there is holding them together. From that calculation we can infer that if there was no more gravity holding the system together than is produced by what we see, then it would fly apart. We can also look at gases and stars in the outlying part of our Galaxy, which orbit around the central hub, and we can conclude that these gases and stars would escape into intergalactic space unless there was more material pulling them in than what we actually see.
The evidence for dark matter itself is simple and almost incontrovertible: the way stars and galaxies are moving suggest that something invisible must be exerting a gravitational pull on them. It's the same reasoning used in the 19th century when the planet Neptune was inferred to exist because the orbit of Uranus was deviated by the pull of a more distant unseen object. In our Solar System, there is a fine balance between the tendency of gravity to make the planets fall towards the Sun, and the centrifugal effect of the orbital motions. Likewise, on the far larger scale of an entire galaxy, there is a balance between gravity, which tends to pull everything together into the centre, and the disruptive effects of motion, which, if gravity didn't act, would make its constituent stars disperse. Dark matter is inferred to exist because the observed motions are surprisingly fast — too fast to be balanced just by the gravity of the stars and gas that we see.
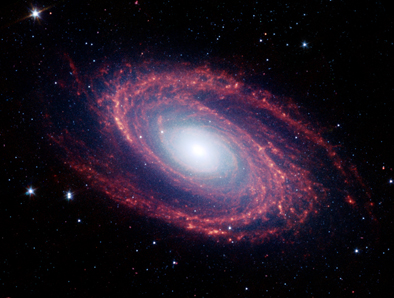
The magnificent spiral arms of the nearby galaxy Messier 81 are highlighted in this NASA Spitzer Space Telescope image. Image courtesy NASA Jet Propulsion Laboratory.
In disc galaxies like our own evidence for dark matter comes from the measurements of the speeds of stars, and of gas clouds. These speeds, particularly those of outliers orbiting beyond most of the stars, far out from the central hub, are puzzlingly high. If the outermost gas and stars were feeling only the gravitational pull of what we can see, they should be escaping, just as Neptune and Pluto would escape from the Sun's influence if they were moving as fast as the Earth does. The high observed speeds tell us that a heavy invisible halo surrounds big galaxies. If there weren't a lot of dark stuff, galaxies would not be stable, but would fly apart. The beautiful discs or spirals are essentially just "luminous sediment" held in the gravitational clutch of vast swarms of invisible objects of quite unknown nature. Galaxies are up to ten times bigger and heavier than they seem. The same argument applies, on a larger scale, to entire clusters of galaxies, each millions of light years across. To hold them together requires the gravitational pull of about five times more mass than there is in the stars and gas we observe.
There are other ways of inferring the presence of dark matter. All gravitating material, whether luminous or "dark", deflects light rays, so clusters can be "weighed" by detecting how strongly they deviate the paths of light rays passing through them. Deflection of starlight by the Sun's gravity, observed by Eddington and others during the 1919 total eclipse, famously offered an early — though in retrospect not entirely convincing — test of Einstein's general relativity. Gravitational bending of light can be understood via a simple analogy with the familiar situation where a concave or convex lens produces a wide variety of images of a candle placed behind it, ranging from highly magnified ones, to demagnified ones, or inverted ones, depending on the precise configuration of the lens and candle. Similarly, the shapes of distant galaxies and quasars viewed through the cluster lens produces a plethora of images ranging from highly distorted arcs to multiple images of the same background object. Knowing the undistorted shapes, the observed ones can be used to infer the mass of the lensing cluster. The mass thus inferred from lensing observations of clusters vastly outweighs that obtained from adding up the light from the stars in the constituent galaxies and that of the hot X-ray emitting gas. This points to the existence of significant amounts of dark matter.
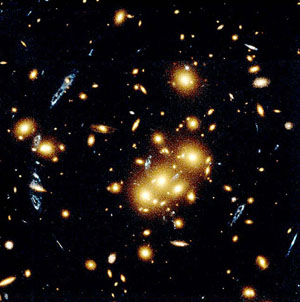
This Hubble Space Telescope image shows several blue, loop-shaped objects that actually are multiple images of the same galaxy. They have been duplicated by the gravitational lens of the cluster of yellow, elliptical and spiral galaxies. Image courtesy NASA Goddard Space Flight Center.
As in the case of galaxies, the picture that emerges for a cluster is one of a rather massive dark matter halo enveloping the gas and galaxies. The Hubble Space Telescope has taken spectacular pictures of several clusters of galaxies lying about a billion light years away. These pictures reveal many faint features, streaks and arcs: each is a remote galaxy, several times further away than the cluster itself, whose image is, as it were, viewed through a distorting lens. We shouldn't really have been surprised to discover that the large-scale motions of galaxies are induced by (or respond to) the gravitational pull of something quite unseen, and that dark matter is the dominant gravitational influence on the cosmos.
There is of course one assumption underlying these inferences of dark matter: namely, that we know the force of gravity exerted by the objects we see. Since the internal motions within galaxies and clusters are slow compared to the speed of light; Newton's inverse square law is valid, which tells us that if you move twice as far away from any mass, the force gets four times weaker. Some skeptics remind us that this law has only really been tested within our Solar System; it is plainly a leap of faith to apply it on scales a hundred million times larger. In fact an Israeli physicist, Mordehia Milgrom, suggested an alternative theory whereby gravity doesn't drop off in strength as much as in Newton's theory. His theory is possible, but it doesn't mesh in so well with cosmology. There's nothing implausible about dark matter per se. Why should everything in the Universe be shining? The challenge is to narrow down the field of candidates and come up with strategies to detect them. I would only take Milgrom seriously if there were no candidates for dark matter. Indeed the problem is that there are too many possible alternatives. Only if these candidates could all be shot down would I be prepared to offer better odds in favour of Milgrom.
What is dark matter made of?
Is it made of brown dwarfs?
Dark matter emits no light — indeed no radiation of any kind that can be directly detected. Nor does it absorb or scatter light. This automatically rules out it being dust. Our Galaxy is very dusty because starlight is scattered and attenuated by intervening clouds that are composed of tiny grains rather like those that produce the haze from tobacco-smoke. But if the grains weighed enough to make up all the dark matter, they would entirely obscure our view of any distant stars.
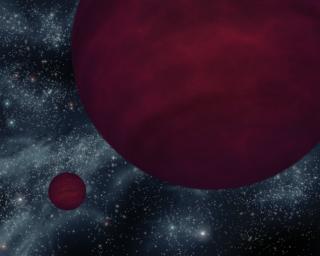
This artist's concept shows twin brown dwarfs referred to as 2M 0939. They are actually the same size but are drawn in perspective. Image courtesy NASA Jet Propulsion Laboratory.
Possible candidates for dark matter are small and faint stars. Stars with below 8% of the Sun's mass are called brown dwarfs. They are not hot enough to ignite the nuclear burning that keeps ordinary stars shining. Brown dwarfs definitely exist: some have been found, as a byproduct of searches for planets, in orbits around brighter stars; others, particularly some nearby ones, have been detected by their very faint emission of red light. The proportions of big and small stars in our Galaxy are determined by very complicated processes that aren't yet completely understood. When stars form today in cosmic clouds, brown dwarfs are outnumbered by bigger stars. If there were enough of them to provide the dark matter, this ratio would need to have been very different in other places or times. For example, the "mix" of big and small stars could have been different when the Galaxy first condensed from primordial gas.
Individual brown dwarfs could reveal themselves by gravitational lensing. If one of them passed in front of a bright star, then the brown dwarf's gravity would focus the light, causing the bright star to appear magnified. As a consequence, a star would brighten up and fade in a distinctive way if a brown dwarf passed in front of it. This, however, requires very precise alignment: such events would consequently be very rare, even if there were enough brown dwarfs to make up all the dark matter in our Galaxy.
However, astronomers have carried out ambitious searches for these microlensing events (called micro to distinguish the phenomenon from the lensing by entire clusters of galaxies already mentioned). Millions of stars are monitored repeatedly, to pick out those whose brightness changes from night to night. Many stars vary for all kinds of intrinsic reasons: some pulsate, some undergo flares, and some are orbiting around binary companions. The searches have found many thousands of these, which are interesting to some astronomers, though a tiresome complication for the microlensing searches.
Occasionally, stars have been found to display the distinctive rise and fall in brightness that would be expected if an unseen mass had crossed in front of them and focused their light. It still isn't clear whether there are enough of these events to implicate a new brown dwarf population, or whether ordinary faint stars, passing in front of brighter ones, are common enough to account for the events recorded. There are several other candidates for dark matter. Cold "planets" moving through interstellar space, unattached to any star, could exist in vast numbers without being detected; so could comet-like lumps of frozen hydrogen; and so could black holes.
Deuterium suggests exotic particles
Brown dwarfs, comets, or even black holes that are the remnants of dead stars are however suspected to be only a minor constituent of dark matter — this is because there are strong reasons for suspecting that dark matter isn't made of ordinary atoms at all. This argument is based on a heavy isotope of hydrogen, called deuterium. It turns out that if dark matter were made from ordinary atoms, then theory predicts that there should be much less deuterium in the Universe than we actually observe.
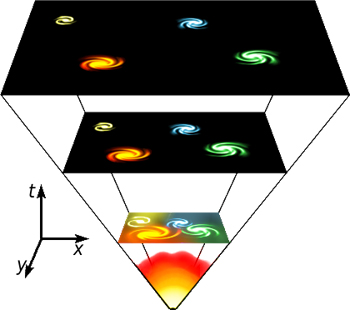
According to the Big Bang model, the Universe expanded from an extremely dense and hot state and continues to expand today.
Any deuterium that we observe must have been made in the Big Bang and not inside stars, as other elements. The actual amount of deuterium in our Universe was until recently uncertain, but astronomers have detected the spectral imprint of deuterium, distinguishing it from ordinary hydrogen, in the light received from very distant galaxies. This measurement has needed the light-collecting power of new telescopes with ten-metre diameter mirrors.
The proportion of deuterium that ought to emerge from the Big Bang depends on how dense the Universe is, and observations agree with theory if there are 0.2 hydrogen atoms in each cubic metre. This proportion agrees quite well with the actual number of atoms in shining objects — half of these atoms are in galaxies, and the other half in intergalactic gas — but nothing much is then left over for the dark matter. If there were enough atoms to make up all the dark matter, the concordance with theory would be shattered. The Big Bang calculations would then predict much less deuterium, and somewhat more helium, than we actually observe: the origin of the deuterium in the Universe would then become a total mystery.
To have less deuterium when the density of atoms is higher at first sight seems a perverse result, but it's actually quite natural. The higher the density, the more often the nuclei hit each other, and the more quickly nuclear reactions convert hydrogen (one proton) into helium (two protons and two neutrons). Deuterium (one proton and one neutron) is an intermediate product. Not much would survive if the density were high, because the reactions would have gone so quickly that nearly all the deuterium would have been processed into helium; on the other hand, if the density were lower, we would expect more "fossil" deuterium left over from the first three minutes of our Universe's existence. The dependence is quite sensitive, so any reasonably accurate measurement of the deuterium fraction tells us the average density of atoms in the Universe. The detected amount of deuterium in our Universe matches the calculations if atoms contribute 4% to what cosmologists call the critical density of the Universe [the critical density ensures a Universe with a flat, rather than curved, geometry, and it's used as a benchmark here].
This tells us something very important: the dominant dark matter is made of something that is inert as far as nuclear reactions are concerned. Exotic particles — not anything made of ordinary atoms at all — make the main contribution to dark matter.
Is dark matter made from neutrinos?
So the nature of this dark matter still flummoxes us. The best guess is that the Big Bang, which we know produced the atoms in the Universe and the heat energy that is manifested as the 3-degree cosmic microwave background radiation, could also have produced other particles. When the Universe was very hot all possible reactions would have happened very fast and therefore produced these particles. What these particles are we don't really know yet, because they require physics that we haven't yet fully formulated. Although we can confidently extrapolate back to when the Universe was a second old, we cannot go any further: the conditions that reigned a split second after the Big Bang were so hot and dense that they fall outside the regime we can actually do experiments on here on Earth. We therefore don't know what kind of particles could have been produced at that stage.
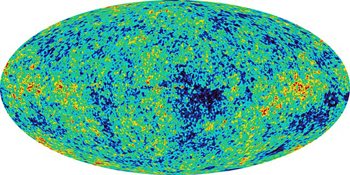
Microwave Sky image from the WMAP Mission, showing a detailed full-sky map of the oldest light in the Universe, dating from 379,000 years after the Big Bang, over 13 billion years ago. Image courtesy NASA Goddard Space Flight Center.
There is however one particle that has been regarded as a candidate for dark matter: the elusive neutrino. Neutrinos have no electric charge, and hardly interact at all with ordinary atoms: almost all neutrinos that hit the Earth go straight through it. During the very first second after the Big Bang, when the temperature exceeded 10 billion degrees, everything was so compressed that the reactions converting photons (quanta of radiation) into neutrinos would have been fast enough to come into balance. As a consequence, the number of neutrinos left over from the "cosmic fireball" should be linked to the number of photons. One can calculate, using physics that is quite standard and uncontroversial, that there should be 340 neutrinos in every cubic centimetre — in other words, hundreds of millions of neutrinos for every atom in the Universe.
Because neutrinos so greatly outnumber atoms, they could make up the dominant dark matter, even if each weighed only a hundred millionth as much as an atom. Before the 1980s, almost everyone believed neutrinos were zero rest-mass particles: they would then carry energy and move at the speed of light, but their gravitational effects would be unimportant. (Likewise, the photons left over from the early Universe, now detected as the cosmic microwave background radiation, don't now exert any significant gravitational effects.) But it now seems that neutrinos may weigh something after all, even though it is a very tiny amount indeed.
The best evidence for neutrino masses comes from the Kamiokande experiment in Japan, which used a huge tank in a former zinc mine. The experimenters studied neutrinos that originate from the Sun (where they are a byproduct of the nuclear reactions in the central core), as well as others that are produced by very fast particles (cosmic rays) impacting on the Earth's upper atmosphere. The experiments imply a non-zero mass, but one that is nonetheless too small to render them important in accounting for much of the dark matter. This discovery about neutrino masses is, nonetheless, important for understanding the neutrinos that come from the Sun. It is also a major advance in physics, since it takes us beyond the so-called standard model of particle physics (see the Plus article The physics of elementary particles).
Is dark matter made from supersymmetric particles?
Although we don't know the exact masses of neutrinos, we at least have evidence that they exist. But there is a long list of hypothetical particles that might well exist, and (if so) could have survived from the Big Bang in sufficient numbers. We might discover these particles (known as supersymmmetric particles) with the new Large Hadron Collider at CERN, but can also look for them in other ways. There are no convincing arguments telling us about how heavy each particle might be: best guesses suggest a few hundred times as heavy as a hydrogen atom. If there were enough such particles to make up all the dark matter in our Galaxy, there would be several thousand per cubic metre in the neighborhood of the Sun. They would be moving at about the same speed as the average star in our Galaxy — maybe 300 kilometres per second.
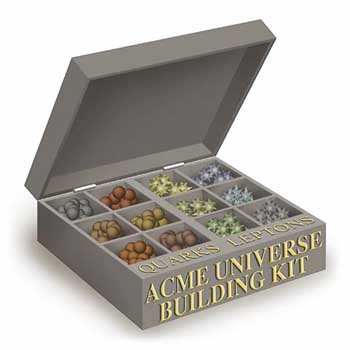
Dark matter may be made of exotic particles which have not yet been discovered.
These particles, heavy but electrically neutral, would generally, like neutrinos, whiz straight through the Earth. However, a tiny proportion might interact with an atom in the material they pass through. There would be only a few collisions per day within each of us (even though our bodies each contain nearly 1029 atoms). We clearly do not feel these hits. However, very sensitive experiments with ultra-pure material as a target can detect the minuscule kick or recoil when such an impact happens, for instance in a slab of silicon, or similar material. The experiment must be cooled to a very low temperature, and carried out deep underground to reduce the confusion from other kinds of event that could drown out any genuine signal from dark matter impacts.
Several groups of physicists have taken up the challenge of this underground astronomy. For example, the Zeplin III project looks for dark matter in an underground mine in Yorkshire, while another project uses the Gran Sasso tunnel under an Italian mountain. It's delicate and tedious work, but if they succeed, they will not only find out what our Universe is mainly made of, but as a bonus may discover an important new kind of particle. Only an extreme optimist would bet more than evens on success. This is because, at the moment, we have no firm theory that tells us what the dark matter particles are, and what properties they might have, and it's hard to focus the search optimally.
Is it made from other particles?
But there are many other ideas for dark matter particles besides the supersymmetric particles. Some theorists favour a type of very light particle called axion. Others suspect that the particles could be a billion times heavier than the predicted supersymmetric particles, in which case there would be a billion times fewer of them, making detection even harder. Or they could be even more exotic still — for instance atom-sized black holes made in the ultra-high pressures of the early Universe.
This is the kind of experiment I am very excited about. It exemplifies how the cosmos offers a chance for us to study "natural" experiments that we could never do in labs here on Earth. There's a symbiosis between astronomy and physics. Astronomers obviously need to know how atoms behave in order to understand the stars — they learn this via laboratory experiments. But now, in a complementary way, astronomers are discovering things which tell physicists something new. We can see where nature has done experiments for us: galaxies crashing together, stars exploding, immensely strong gravity. If the people at Gran Sasso (or at any of the other laboratories around the world engaged in this search) detect the dark matter, it will be a great triumph. Not only because they will detect what much of the Universe is made of, but because they will also detect a new type of particle, which will be a more important discovery than that of the cosmic microwave background radiation by Penzias and Wilson in 1965.
So what's the likeliest candidate for dark matter?
There are probably several different kinds of dark matter. It would, for instance, be surprising if there weren't some brown dwarfs and black holes. However, exotic particles seem far more likely, There is an entire zoo of particles already known to science, and no doubt many that remain undiscovered. When the physics of extreme energies and densities is better understood, we should know what other kinds of particles might once have existed, and be able to calculate how they would have survived from the first millisecond just as confidently as we can now predict the amount of helium surviving from the first three minutes.
But for the moment, we still don't know what dark matter is. Together with its mysterious companion dark energy it makes up 95% of our Universe. This means that 95% of the Universe is unaccounted for. We're coming to terms with the inference that atoms, planets, stars and galaxies — everything we're made of and everything we can see — amount to less than 5% of all that's there. Cosmic modesty has to be taken a step further: we've gradually learnt, ever since Copernicus, that we're not located at any special central place in the cosmos; now it turns out that we're not made of the dominant stuff — atoms seem a mere afterthought in a Universe whose structure and fate are controlled by quite different and mysterious substances. The dark side controls our Universe — how it began, its eventual fate, and whether it's finite or infinite.
Comments
Anonymous
i think some particles heavier than axions,neutrinos,etc; are there called wimbs yeah but i am not sure . well nobody is.
Anonymous
http://en.wikipedia.org/wiki/Weakly_interacting_massive_particle
Anonymous
Science is struggling to determine what dark matter is and in most cases people are assuming dark matter was formed during the big bang. It is my belief that dark matter is largely undetectable as the focus for the search uses techniques based on assumptions that our universe in its entirety was formed during the big bang. The thought that nothing existed apart from one supper dense ball of elements prior to this event is laughable. We already know that density of an object has little effect on neutrinos and our principle theory for dark matter is that it is some form of neutrino. It is also unhelpful to refer to dark matter as one thing or one type of element as we haven't discovered what it is yet. More likely is that dark matter is made up from as many or even more element known by us and is in as much abundance as the matter we know of. Until people are willing to let go of an out dated theory because it satisfies known physics principles we will never discover dark matter.
I leave you with a thought. Why do objects with mass have gravity? (Saying because we have witnessed it is not an answer). 2D physics will not solve a 4D problem!
James Mewis
Jackfield, UK
Anonymous
I am a mechanical engineer with a love for theoretical physics. I agree with your comment. Sometimes I feel are stuck thinking in the dimensions we can perceive. Which is why we call Dark Matter "Dark" - we can't perceive it with the way we currently think. Although we should not think in 2D to solve a 4D problem, it may actually help us visualize it. I will explain in a minute. As an "outsider looking in", what I can see is that Dark Matter really is all about 4D - and even higher D's. I've read in more than 1 occasion that our Universe can allow up to 11 dimensions. I believe we all can agree that the presence of Mass on the fabric of space-time is what causes gravity - and that space-time bends. Therefore, there has to be more "room" to allow for the bending.
In one of my Materials Engineering courses, I remember the term "Elastic Limit". In essence, when a material is stressed to the point of exceeding its elastic limit, there will be a permanent deformation. For example, if we had a sheet of a rubber-band like material stretched out and put a lead ball in the middle, it will deflect. Once the ball is removed, it will return to it's original shape. Now, let's imagine something like a flat sheet of Aluminum foil. Imagine if we put that same lead ball. If the sheet is stretched taught, not only will it bend/deflect, there may be a permanent deformation. This may not be the best example, but we can visualize this. So - what is left behind? A sheet with a curved, dimple on it. There is nothing there, but we would see and feel the consequence of that deflection if we where " tiny 2D beings" living on that sheet.
Therefore, what if what we are perceiving as Dark Matter really is only a "permanent deformation" of the fabric of Space-Time? Could it be that something extremely massive existed there at some point - and moved on?
Maybe in a "previous life" of our universe, there was something massive there and left its "impression". If we manage to detect what is really happening to space-time at those points, we will have an idea of what Dark Energy is as well. After all, the energy-matter has to balance.
-Fernando
Tarrytown, NY
Anonymous
I belive your explanation is greatly thought out and a 10 year old could understand it(brefly...). also I like your idea of dark matter and the whay you precive it, I havea similar theory. Thankyou this will help me explain(and understand) dark matter.
Anonymous
Nothing pops up without raw material. Everything we see and touch is a compounded matter and an attribute of compounded matters is the life span its nature allows it to exist. How did the universe arrived to its present size when it used to be just a dot. That dot was composed of solid material or element. It exploded due to the imbalance in density between the solid dot and atmosphere around it. As the fragmentations resulting from the explosion are propelled and spin they draw the elements enveloping them; these elements are what we refer as dark matter. But where did the dark matter come from? When the life span of a celestial body ends it would release the elements composing it. These elements are the same elements that the next arising body would be made of. Dark matter is everywhere including earth. The mushroom that spring during thunderstorm are the same dark matter that celestial bodies are made of.
On the other hand, dark energy is explained by atomic vibration. The mechanism that makes an atom to vibrate is the same mechanism that holds celestial bodies together. The key in understanding dark energy is the fact that size is a matter of number, meaning the large the body the more atom composing it. An imperceptible atom is made of few number of particles compared to a galaxy that is composed of countless number of atom. Size does not matter, the law governing an atom and a galaxy is the same. The only difference is magnitude, i.e., small atom vibrates rapidly and a galaxy has immeasureable vibration (life span).
For more detail pls visit josephtarellano.blogspot.com, "why global warming is unstoppable".
Anonymous
So I was wondering - when someone travels in time to another-then, would they also transport all of their micro-organisms along for the ride? Would their last meal from the other-then still be digesting in their gut, to be deposited in some bathroom within this-then? Would they have disturbed some delicate mass-energy balance equation by bringing along a snack from the other-then to this-then, and by eating it in this-then and convert it into heat-energy cause some backlash yinyang/ karma balancing process to kick-in? Would an entering time-traveller have to displace, say one-Tardis-full unit of 3D space/time/air/mass from its current location to somewhere-else/ somewhen-else? Would the same amount of mass/ energy need to be replaced upon exiting? Could this time-traveling mass-energy mis-matches be the origins of the "dark-energy/ dark-matter" that we are currently detecting in the this-then? Is the overall effect cumulative/ recursive?