
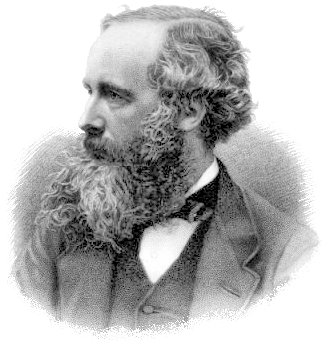
James Clerk Maxwell (1831-1879).
2015 is a year of anniversaries. We've celebrated the centenary of Einstein's general theory of relativity, and the 200th birthday of George Boole, whose logic powers modern computers. But there's also a third anniversary — and it's as relevant to modern technology as it is to our understanding of the Universe.
Almost exactly 150 years ago the Scottish physicist James Clerk Maxwell worked out how to combine electricity and magnetism, two things that at first seem unrelated. In 1865 Maxwell published a set of equations that describe all electromagnetic phenomena, as they are called. The name might sound fancy, but we come across these phenomena every day of our life: in the form of the visible light we rely on to see the world around us, the TV and radio that keep us entertained, and the wifi or mobile phone signals that keep us connected. It's almost impossible to list all the areas of physics and technology the equations are useful in.
Catching waves
Taken on their own, electricity and magnetism have been known for a very long time. "The words 'electricity' and 'magnetism' go back to the ancient Greeks," explains John Ellis, Clerk Maxwell Professor of Theoretical Physics at King's College, London, where Maxwell himself was a professor. "People knew about these phenomena, but it wasn't really until the 18th, and particularly the early part of the 19th century, that they realised there must be connections between them." By the middle of the 19th century experimentalists, including Michael Faraday, had established clear evidence for the connection. They had shown, for example, that electric currents can generate magnetic fields, and that moving magnets can generate electric currents.
"There were a number of different theoretical ideas, and Maxwell came in and made sense of everything," says Ellis. "He showed how to describe electricity and magnetism in a connected way. He showed that many of the previous ideas were [rubbish]. And he discovered some very remarkable things."
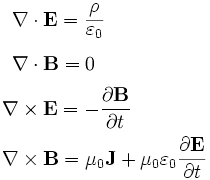
These are Maxwell's equations. You can find a nice explanation of them here.
One of Maxwell's remarkable predictions relates to the electric and magnetic fields mentioned above. These aren't necessarily static, but can experience regularly undulating changes that propagate like waves through space. Maxwell's equations predict that these oscillations of electric and magnetic fields are interlocked: leading to the idea of electromagnetic waves that propagate through space at very high speed.
"When people hear about waves, they [usually] think about water waves, or maybe sound waves," says Ellis. "[Electromagnetic waves] are rather more abstract, but they have very concrete consequences. Actually, these are what we understand as light and radio, [etc]. All those things are electromagnetic waves, which were predicted by Maxwell on the basis of his equations."
The equations also enabled Maxwell to calculate just how fast those electromagnetic waves travel through empty space, and to answer, thereby, a question people had been contemplating for a long time. "It was known previously that light, although it travels very, very, very fast, doesn't travel infinitely quickly," explains Ellis. "It takes some time to get from A to B, and various experiments [had] measured this. I think one of the most remarkable eureka moments in scientific history must have been when Maxwell sat down and calculated, from his equations, the speed of light, and got the right answer." (The answer is nearly 300,000,000 metres per second.)
The result was encouraging, but it took almost another 25 years until the physical existence of the waves predicted by Maxwell was proven in experiments. "Heinrich Hertz demonstrated the physical reality of these waves by generating an oscillating electric current, and then picking up a signal corresponding to what we would now talk about as radio waves in a receiver," explains Ellis. "He just did that in a lab, so perhaps you might say it was a bit of a curiosity. But then, shortly afterwards, Guglielmo Marconi showed that you could send radio waves across the Atlantic. That really revolutionised the way that human beings communicate — all that goes back to Maxwell's equations."
Unification
The practical uses of Maxwell's equations are impressive, but many physicists celebrate their anniversary for a more fundamental reason: the equations brought us closer to understanding the nature of the world we live in. "If we look around in the Universe today, it's quite complicated." says Ellis. "But we physicists are in the business of trying to understand how it works and how it got to be the way it is today. So we try to look for connections between different phenomena, some sort of underlying cause. That is [called unification]. We try to unify our descriptions of various different pieces of nature. It's intellectually satisfying to understand the connections between different things that are happening in the Universe. But, also, as the example of these electromagnetic waves indicates, it enables you to do things that you just couldn't imagine doing previously."
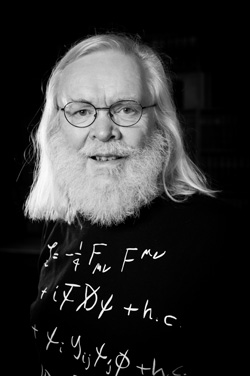
John Ellis.
Fundamental physics has come a long way since Maxwell's time. By the late 1930s people had realised that there were other fundamental forces besides electromagnetism and the force of gravity (which had been described by Isaac Newton in the 17th century and by Albert Einstein in 1915). They discovered the strong nuclear force, which holds together atomic nuclei, and the weak force, which is responsible for some forms of radioactive decay. "The next piece of business for the 20th-century physicists was to see if they could understand these other fundamental forces," says Ellis.
To describe the weak force, physicists drew analogies to electromagnetism, and eventually found themselves a step higher up the unification ladder. Their ideas suggested that the two forces were, in fact, just two sides of the same coin: the unified electroweak force. It's a curious idea because the weak force doesn't behave either like electricity, or like magnetism. For starters, and as its name suggests, it's a lot weaker. It only acts over a tiny range of 3 x 10-17 metres, and at the nuclear scale the weak force is 10,000 weaker than the electromagnetic force. "If it wasn't weak, life would be impossible," says Ellis. "It's not that we'd all glow in the dark, we would never [even] be born to begin with. The Universe would just be completely different if that weak force were not weak."
The idea of unification suggests that the similarity of the two forces, electromagnetism and the weak force, was only apparent right after the Big Bang, when the Universe was incredibly hot. As temperatures cooled down, the forces crystallised out and became different. Weird as it might seem, the concept isn't entirely unfamiliar: think of the dramatic change that happens to water when it freezes to ice.
In the 1960s various physicists pieced together a theory that described both forces, the electromagnetic and the weak force, in one unifying mathematical framework. "The underlying description of these forces is very much like Maxwell's, so that's unification," explains Ellis. "It's a more complicated set of equations, but, in principle, they're relatively simple, because there's a symmetry that relates them together."
The difference between the forces, as we see them today, was explained via a process that caused the symmetry to go into hiding. Water again gives a good analogy for this. The laws of nature responsible for the behaviour of water are the same everywhere and they don't favour any particular direction in space — which is why a patch of ocean looks much like any other, and appears the same no matter from what direction you look at it. The icebergs that form when the water freezes, however, display none of that symmetry: no two will look the same, and you'd have to be incredibly luckily to find one with rotational symmetry. The symmetry of the theory — its indifference to place or direction — simply isn't manifest in individual outcomes. But it's still there, hiding in the background.
Going back to forces, it turns out that each force is carried across space by messenger particles called bosons. Initially, all messenger particles (indeed all particles in the Universe) started out having no mass at all. But as the Universe cooled down, things "froze" into different shapes: the messenger particles of the weak force (and other particles) acquired mass, while the messenger particles of electromagnetism remained massless. The heaviness of the weak bosons means they are hard to produce, and that's what renders the force weak. "If those particles weren't heavy, then the weak force would be as important as electricity and magnetism, and we'd all be fried," says Ellis. (See here for more about the physics of elementary particles.)
Discovering new particles
The theory attracted little attention at first, but over the 1970s theoretical as well as experimental results began firming it up. "I got into the game in 1975, because I said, 'Well, look. Obviously, these [heavy messenger particles of the weak force] have to exist, so somebody will find them eventually.', " says Ellis, and he was right. The heavy messenger bosons of the weak force (called Z and W bosons) were discovered at CERN in 1983. "But the really key aspect of the whole thing [was] this object called the Higgs boson. This particle [is in some sense] the messenger of this breaking of the symmetry in the unified theory of electricity and magnetism and the weak interactions. So Mary Gaillard, Dimitri Nanopoulos and I wrote a paper discussing what this particle would look like. The Higgs boson later became, in some sense, the holy grail of particle physics. Finally, in 2012, experiments at the LHC discovered it, and so completed this picture of, on the one hand, unification, and, on the other hand, symmetry and how it's broken." (You can read more about the Higgs boson here.)
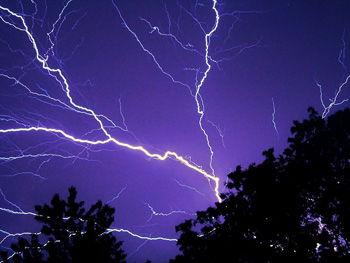
Lightning is electrostatic discharge during an electrical storm between electrically charged regions of a cloud.
Electroweak unification was a real triumph of theoretical physics. It resulted in a Nobel Prize in Physics for Sheldon Glashow, Abdus Salam, and Steven Weinberg (for the unified electroweak framework), and for François Englert and Peter Higgs (for the description of the mass-related symmetry breaking mechanism). Like Maxwell and Ellis, Higgs too spent time at King's College, London, though as a student rather than professor.
The hunt for unification, to which Maxwell so greatly contributed, is far from over. Ideally, physicists would like to show that all forces, including the strong nuclear force and the force of gravity, were once one and the same and only broke apart as the Universe cooled down from the Big Bang. It's a daunting task — gravity especially is proving a major challenge in this grand unification scheme.
In the meantime, can we hope for the practical benefits of theoretical research? "Nowadays, governments often like to fund research in a directed way," says Ellis. "They would like to have a better widget and so they pay people to produce a better widget. I think Maxwell's equations and the story of the electromagnetic wave are the perfect example that, actually, the most revolutionary discoveries do not come about because you're looking for a better widget. Often, fundamental discoveries in physics, steps forward in unification, turn out to have completely unexpected and really revolutionary ramifications [in technology]."
"These stories about [things like the] Higgs boson show you that mathematical physics has incredible powers of prediction. You write down your equations, you understand the symmetries of those equations, and they give you tremendous predictive power. I'm not aware that, in any other domain of human endeavour, you have the same power."
About this article
John Ellis is Clerk Maxwell Professor of Theoretical Physics at King's College, London. Marianne Freiberger is Editor of Plus. She interviewed Ellis in November 2015, following a discussion meeting at the Royal Society which celebrated the anniversary of Maxwell's equations.