
The 2021 Nobel Prize in Physics has been awarded to Giorgio Parisi, who receives one half of the Prize, and Syukuro Manabe and Klaus Hasselmann, who share the other half. The three have been awarded for their work in helping us understand extremely complex processes and systems — and in all three cases it's maths that has made the breakthroughs possible.
From weather to climate
When, in 1961, the meteorologist Edward Lorenz was using computer models to simulate the weather, he accidentally discovered the phenomenon of mathematical chaos. Lorenz had come up with a set of equations that described the bare bones of atmospheric processes and his computer was chugging through the calculations. One day he decided to run a particular simulation, based on a particular set of starting values, once again. To his surprise Lorenz saw a weather pattern emerge that was wildly different from what he had observed in his first run. Upon checking he realised the problem was down to a tiny inaccuracy in the numbers he had used as starting values for the second run: rather than 0.506127, which is what the computer had been working with during the first run, he had used 0.506. In the many repeated calculations this tiny error had snowballed to dramatic effect.
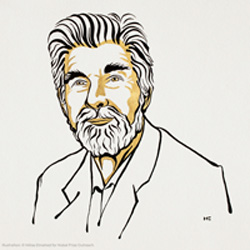
Klaus Hasselmann © Nobel Prize Outreach.
What Lorenz discovered is now known as the butterfly effect — even the tiny disturbance caused by the flap of a butterfly's wing can grow into a tornado halfway around the world. Lorenz' system of equations suffered from a sensitive dependence on initial conditions which meant that slightly different starting conditions could yield a vastly different outcome. The discovery explains why it's impossible to reliably predict the weather for more than a few days into the future.
Karl Hasselmann received his quarter of the Nobel Prize in Physics for creating a model that links those chaotic and rapidly-changing weather phenomena to the long-term predictions for the Earth's climate, which varies more slowly. His inspiration came, not from meteorology, but from a classic example of random behaviour: Brownian motion, named after the Scottish botanist Robert Brown, who in 1827 looked at pollen grains suspended in water under a microscope and found them moving in a highly irregular fashion.
This jittery motion was independently explained by Albert Einstein and Marian Smoluchowski in 1905: the vibrating fluid molecules in the liquid give tiny kicks to the microscopic particles which accumulate to buffet the microparticles about. The resulting Brownian motion is random — you can't predict with certainty the position of one of these microparticles from one moment to the next — but you can assign a probability distribution describing where a particle might move to. This gives you a mathematical handle on the overall behaviour of the system of particles — and as it turns out, the same type of random motion turns up in many other contexts too (see here to find out more).
By analogy, Hasselmann thought of climate and weather as heavy particles (climate) embedded in an ensemble of much lighter particles (weather), which bump into the heavier ones, exerting forces on them. Using the mathematical theory of Brownian motion Hasselmann constructed a stochastic climate model, which incorporates a degree of randomness, and links the variability of the climate to random forcing by short-term weather components. This put climate modelling on a firmer scientific footing and also showed that rapid changes in the atmosphere can lead to long-term changes in the oceans.
After Hasselmann had completed his model for how the climate changes, he also developed ways of spotting the impact of human actions on climate within the model. As this lovely article on the Nobel Prize website describes, "[he] thus cleared the way to further studies of climate change, which have demonstrated traces of human impact on the climate using a large number of independent observations."
The effect of carbon dioxide
Hasselmann shares one half of the Nobel Prize with Syukuro Manabe, whose work has shed light on the impact of carbon dioxide on the Earth's climate.
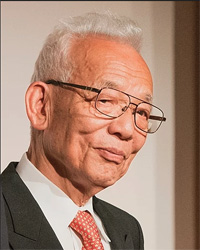
Syukuro Manabe in 2018. Photo: Bengt Nyman, CC BY 2.0.
The fact that increased concentrations of carbon dioxide can lead to global warming can already be gleaned from an astonishingly simple energy balance model we explored in a previous article. The model is based on the assumption that the energy that arrives on Earth from the Sun is equal to the energy that is radiated back out into space. It contains a parameter called the emissivity, which measures how transparent the Earth's atmosphere is: the lower the emissivity, the less energy radiates back out. Since greenhouse gases like carbon dioxide reduce the emissivity, they also lead to an increase in the Earth's temperature (see here for the details).
While instructive, the basic energy balance model makes a number of rash simplifications. For example, it assumes a uniform temperature throughout the Earth. When Manabe started to work on climate in the 1960s he was after more sophisticated models focussing, not just on radiation balance, but also including the vertical movement of air masses due to convection (which causes hotter materials to rise and colder ones to sink) as well as the latent heat of water vapour, the most powerful of greenhouse gases.
Since the calculations involved in understanding these processes would have required a horrendous amount of computing power, which wasn't available at the time, Manabe made a drastic but useful simplification. He imagined the Earth's atmosphere as a one-dimensional column extending 40km upwards. The predictions of the model he built on this simplification confirmed the role of carbon dioxide in a heating of the atmosphere. Manabe eventually extended it to three dimensions and published the result in 1975. This, according to the article on the Nobel Prize website, constituted "yet another milestone on the road to understanding the climate's secrets."
"Syukuro Manabe and Klaus Hasselmann have contributed to the greatest benefit for humankind, in the spirit of Alfred Nobel," the article goes on to say. "We can no longer say that we did not know — the climate models are unequivocal. Is Earth heating up? Yes. Is the cause the increased amounts of greenhouse gases in the atmosphere? Yes. Can this be explained solely by natural factors? No. Are humanity's emissions the reason for the increasing temperature? Yes."
Complex materials
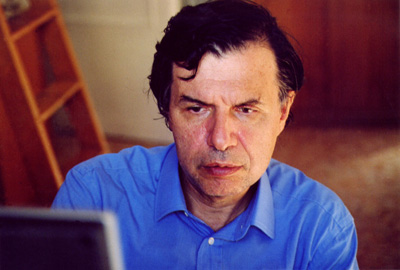
Giorgio Parisi.
While Hasselmann was using particles as metaphors in his study of weather and climate, Giorgio Parisi considered them in the literal sense: as the components that materials are made of. The link between the smallest constituents of, say, a liquid or gas and the substance as a whole was first illuminated in the 19th century. It was realised then that properties such as temperature and pressure are a result of the random motion of those tiny constituents. Following, or predicting, the individual motion of all these tiny particles would of course be impossible, there are too many of them, but luckily it's possible to say things about their bulk behaviour: for example, temperature is the average value of the kinetic energy of all the particles in a material. The field of statistical mechanics makes the invaluable link between the microscopic and macroscopic scale.
The microscopic view of materials also explains what happens when water freezes to ice, or a material becomes magnetised. Individuals particles stop their vigorous jostling about and settle down in a more ordered structure. In the case of ice, this involves water molecules sitting in a hexagonal lattice (see here). In the case of a magnet it involves many individual particles, which each have their own magnetic field, lining up with all their magnetic fields pointing in the same direction (see here).
The materials which Parisi considered, though, are more tricky than ice or an ordinary magnet. Spin glasses also contain iron atoms, each behaving like a little magnet, but these are randomly mixed into a grid of, for example, copper atoms. Rather than aligning their magnetic fields, the iron atoms are frustrated in this case: some pairs want to align, but others want to point in opposite directions. The question is, how do these frustrated atoms settle into an equilibrium?
Parisi provided the answer in 1979 using a mathematical technique called the replica method. Very loosely speaking, this ingenious trick enables you to calculate information about the statistical properties of a system (such as a spin glass) by computing certain average quantities over many replicas of the same system, which might be easier to work out. Although it took people a very long time to prove that Parisi's solution is correct, it has now been used to understand other kinds of disordered systems and has become essential in the theory of complex systems.
"Parisi's fundamental discoveries about the structure of spin glasses were so deep that they not only influenced physics, but also mathematics, biology, neuroscience and machine learning," says the article on the Nobel Prize website. "All these fields include problems that are directly related to frustration."
At first sight, weather and climate modelling appear to have nothing to do with material science. Look at these field more closely, though, and you'll find that in all cases complexity can arise from the interaction of comparatively simple processes — this is why Perisi, Hasselmann and Manabe share this year's Nobel Prize in physics. Congratulations!